Mohamed S. Rashed,1* Martin P. Bucknall,1 Douglas Little,1 Amin Awad,1
Minnie Jacob,1 Mohamed Alamoudi,1 Mona Alwattar,1 and Pinar T. Ozand1,2
Metabolic profiling of amino acids and acylcarnitines from blood spots by automated electrospray tandem mass spectrometry (ESI-MS/MS) is a powerful diagnostic tool for inborn errors of metabolism. New approaches to sample preparation and data interpretation have helped establish the methodology as a robust, high-throughput neonatal screening method. We introduce an efficient 96-well-microplate batch process for blood-spot sample preparation, with which we can obtain high-quality profiles from 500-1000 samples per day per instrument. A computer-assisted metabolic profiling algorithm automatically flags abnormal profiles. We selected diagnostic parameters for the algorithm by comparing profiles from patients with known metabolic disorders and those from normal newborns. Reference range and cutoff values for the diagnostic parameters were established by measuring either metabolite concentrations or peak ratios of certain metabolite pairs. Rigorous testing of the algorithm demonstrates its outstanding clinical sensitivity in flagging abnormal profiles and its high cumulative specificity.
INDEXING TERMS: amino acids•organic acidemia•defects of fatty acid oxidation•inherited disorders•neonatal screening
Inborn errors of metabolism (IEMs)3 and other inherited Mendelian disorders are common in Saudi Arabia and throughout the Middle East, presumably because of the relatively high rates of consanguinity [1–4]. Even in segregated communities, IEMs are estimated to account for as much as 20% of disease among full-term neonates not known to have been at risk and may affect as many as 1 in 5000 live births [5, 6]. Many of the IEMs carry serious clinical consequences to the affected neonate or young infant, including mild or severe mental retardation, physical handicap, and even fatality. Although early diagnosis for some of these disorders has proven very effective in treatment or management, neonates are screened for only a handful of diseases, even in the developed world [7–11].
For>30 years in developed countries, the Guthrie qualitative bacterial inhibition test for phenylalanine in blood spots has formed the basis for successful national neonatal screening programs for phenylketonuria (PKU)[12]. Similar methods have been adopted on a limited scale for diseases such as maple syrup urine disease, isolated hypermethioninemia, homocystinuria, and galactosemia [10, 13]. The more comprehensive quantitative determination of plasma amino acids by HPLC is usually reserved for selective screening of high-risk infants, for confirmation of neonatal screening results, for follow-up of treatment, and for identification of other aminoacidopathies [14, 15].
3 Nonstandard abbreviations: IEMs, inborn errors of metabolism; PKU, phenylketonuria; CAMPA, computer-assisted metabolic profiling algorithm; ESI-MS/MS, electrospray tandem mass spectrometry; FAB-MS/MS, fast atom bombardment tandem mass spectrometry; FDI, functions of diagnostic interest; MDI, masses of diagnostic interest; RDI, (peak) ratios of diagnostic interest; and GA-I/II, glutaric acidemia type I/II.
Organic acidemias caused by mitochondrial enzyme defects in the catabolism of branched-chain amino acids and fatty acid oxidation defects constitute a group of .20 disorders in which acyl-CoA esters accumulate in the mitochondria. In these disorders, carnitine plays a key role, removing the potentially toxic acyl-CoA esters through the formation of acylcarnitine esters and thereby releasing coenzyme A and restoring mitochondrial homeostasis [16, 17]. This results in increased concentrations of circulating acylcarnitines, increased excretion of acylcarnitines in urine, and secondary carnitine deficiency [18]. Therefore, metabolic profiling of free carnitine and acylcarnitine in plasma, blood spots, or urine by various spectrophotometric, radiochemical, or mass-spectrometric methods provides a powerful selective screening tool for these disorders [19–25].
However, the above methods for either neonatal or selective screening of metabolic disorders share some, if not all, of the following drawbacks: (a) narrow spectrum of diseases covered by each method; (b) high falsepositive rates; (c) low specificity or sensitivity (or both); (d) laborious sample preparation; and (e) long analytical time. Millington et al. put forward the concept of broadspectrum screening for IEMs by fast atom bombardment tandem mass spectrometry (FAB-MS/MS) for metabolic profiling of acylcarnitines from plasma or dried blood spots [26–28]. MS/MS, by eliminating the need for chromatographic separation, shortens analysis time to ,2 min, thereby offering the possibility of high sample throughput. The specificity and the accuracy of the technique in profiling amino acids from blood spots have previously been demonstrated in diagnosis of such diseases as PKU, maple syrup urine disease, and homocystinuria [29–31].
The introduction of electrospray tandem mass spectrometry (ESI-MS/MS) to this area of biochemical diagnostics has offered a robust and more sensitive alternative to FAB-MS/MS [32]. Automated ESI-MS/MS is specific and accurate, with real potential as a high-throughput method of screening for many IEMs [33, 34]. Moreover, the technique has been successfully applied to prenatal diagnosis of several organic acidemias from amniotic fluid and to postmortem diagnosis from bile spots [35, 36]. Our objectives in the current study were to develop and evaluate a microplate-based batch method for preparing blood-spot samples, and to develop a computer algorithm for automated flagging of abnormal metabolic profiles by using MS/MS-derived diagnostic parameters for amino acids and acylcarnitines in newborns.
Materials and Methods
computing environment
All computers used in this study were Intel-based personal computers (PCs) running either Microsoft Windows for Workgroups or, more recently, Microsoft Windows 95. These PCs are connected in a peer-to-peer local area network. All in-house program development was carried out with Microsoft Visual Basic Professional and Microsoft Access.
chemicals
Acetyl-[d3-methyl]carnitine, propionyl-[d3-methyl]carnitine, octanoyl-[d3-methyl]carnitine, and palmitoyl-[d3- methyl]carnitine were generous gifts of David Millington (Duke University, Durham, NC). [d3-Methyl]carnitine, 1-[13C,15N]glycine, alanine-2,3,3,4-d4, valine-d8, leucine-5,5,5-d3, methionine-d3, ornithine-3,3,4,4,5,5-d6, phenylalanine-ring-d5, and tyrosine-ring-d4 were purchased from Cambridge Isotope Labs. (Woburn, MA). Butanolic HCl was prepared by saturating dry HPLC-grade 1-butanol with HCl gas for 60 min. HPLC-grade methanol and acetonitrile were purchased from Fisons Scientific Equipment (Loughborough, UK). “Water for Irrigation” was purchased from Abbott Labs. (N. Chicago, IL).
blood samples
The blood samples used in this study were collected according to the guidelines of our Research Council. The samples were taken from newborn babies in several hospitals throughout Saudi Arabia and from sick children referred to our metabolic unit. Blood collected by heelstick was applied to four marked circles on barcoded Guthrie cards (made of S&S 903 filter paper; Schleicher and Schu¨ ll, Dassel, Germany), allowed to dry, and then returned to our laboratory by mail or courier service. Upon receipt, the barcode number for each sample was read by a barcode reader (from PSC, Webster, NY), and the patient’s medical record number and biodata were logged into our database.
microplate batch preparation
We process samples in batches of 96 (or fewer), in standard 96-well U-bottomed microplates (capacity 300–400 mL per well). An in-house-developed automated blood-spot puncher punches a single 3/16-in. (;4.75 mm) blood spot from each Guthrie card directly into the individual wells of a 96-well microplate. An in-house software program written in Microsoft Visual Basic, together with a PSC barcode reader, is used to read the sample ID codes and to map them to the plate positions as each blood spot is punched. To allow for tracking in our system, a file name is given to each batch (microplate). We prepare the samples as previously described [33], but with some modifications. To the blood spot punch in each well we add a methanolic solution (100 mL) containing known concentrations of stable isotope-labeled standards of the following compounds: 1000 pmol each of glycine and alanine; 250 pmol each of valine, methionine, and phenylalanine; 500 pmol each of leucine and tyrosine; 221 pmol of ornithine; 125 pmol of carnitine; 60 pmol of acetylcarnitine; 6 pmol of propionylcarnitine; 6 pmol of octanoylcarnitine; and 12.5 pmol of palmitoylcarnitine. We dispense the methanolic solution with an Eppendorf Repeater 4780 pipette (Eppendorf-Netheler-Hinz, Ham-
burg, Germany), which uses disposable 1.25-mL “combitips” to deliver several 100-mL aliquots from a single filling. The microplate is covered with aluminum foil, and the samples are extracted for 30 min at 4 °C. The methanolic extracts are then transferred with an 8-tip multipipettor to identical positions in a hexane-resistant vinyl U-bottomed microplate (200-mL capacity per well; Costar, Cambridge, MA). The methanol is removed by heated evaporation at ;50 °C under an electrical hot-air blower. To the residue in each well we add 50 mL of butanolic HCl, again using the Repeater device, and seal the microplate with self-adhesive tape (from Manco, Westlake, OH); derivatization is completed by heating at 65 °C for 20 min. Excess butanolic HCl is removed with the hot-air blower, and the whole plate is washed by immersion in hexane for 1 min; we then shake the hexane out of the wells by tapping the inverted plate on a hard surface and let the plate dry at room temperature. We reconstitute the final residues in 125 mL of mobile phase (see below), again covering the plate with aluminum foil (to prevent evaporation), and place the plate in the autosampler tray for injection into the mass spectrometer.
sample introduction and mass spectrometry
We use a Model PU-980 HPLC pump and a Model AS-950 autosampler (both from Jasco International Co., Tokyo, Japan) for solvent delivery and automated sample introduction. The injector outlet port is connected via a length of fused silica to the inlet of the electrospray-equipped VG Quattro triple-quadrupole mass spectrometer (VG Biotech, Fisons Instruments, Altrincham, UK). Acetonitrile: irrigation water (80:20 by vol) is used as mobile phase and to flush the autosampler syringe between injections. The autosampler is fitted with a 20-mL loop and is programed to overfill the loop (fill volume 26 mL) directly from each well. For all our routine analyses, the HPLC pump is programed to give a flow rate of 17 mL/min. Injections are made at 2.7-min intervals, a contact closure signal being generated at injection to start the event cycle of the HPLC pump. At 0.3 min after injection, the pump gives a contact closure output, which starts the mass spectrometer’s data-acquisition sequence. This postinjection delay allows time for the sample to reach the ion source. The pump is programed to momentarily increase the flow rate to 1.0 mL/min at 2.4 min after injection. This flow surge at the end of the injection cycle serves to wash the sample loop and clears any residual material from the electrospray interface of the mass spectrometer. The remaining 0.3 min of the cycle allows reequilibration of the liquid flow rate to 17 mL/min before the next injection. The electrospray source temperature is maintained at 120 °C, and the pressure of argon gas inside the collision cell is adjusted to 0.4 Pa. Nitrogen from a cylinder, regulated to 103 kPa, is used as nebulizing gas, and compressed air from the in-house supply, regulated to 300 kPa, is the bath gas. We set the resolution of both mass spectrometers, MS1 and MS2, to give peak widths of 1.2 Da at 15% height and set the instrument’s ion counting threshold to 5.
We use a program developed in-house to retrieve the plate map from the centralized database and to transpose the data into a compatible format for use as a sample list for the mass spectrometer’s Masslynx software. The program places the formatted list onto the Windows clipboard of the mass spectrometer’s PC for later pasting into the Masslynx sample list editor. A project file created in the Masslynx software is given the same unique name as that of the plate. The plate map list is pasted (from the clipboard) into the sample list editor of the project file and saved.
For all routine work, we programed the mass spectrometer to acquire each patient’s sample as a separate data file in the multichannel analysis mode. The following 1.9-min sequence of four MS/MS positive-ion scan functions is initiated by a contact closure from the HPLC pump: (a) free carnitine and acylcarnitines (0.00–1.00 min): precursor ions of mass 85, from 215 to 500 Da, scan time 3.0 s, cone voltage 28 V, collision energy 25 eV; (b) amino acids (1.00–1.45 min): neutral loss of 102 Da from 125 to 300 Da, scan time 0.95 s, cone voltage 20 V, collision energy 10 eV; (c) amino acids (1.45–1.65 min): neutral loss of 119 Da from 150 to 270 Da, scan time 0.75 s, cone voltage 20 V, collision energy 25 eV; and (d) amino acids (1.65–1.90 min): neutral loss of 56 Da from 120 to 155 Da, scan time 0.4 s, cone voltage 20 V, collision energy 8 eV.
At the end of the 1.9-min data-capture cycle, the file name for the next sample is loaded, and the mass spectrometer is reinitialized to receive a new acquisition start signal from the HPLC pump. The time difference between the injection intervals of 2.7 min and the mass spectrometer acquisition time of 1.9 min allows for purging the system, injecting the sample, and letting the sample reach the ion source of the mass spectrometer.
evaluation of microplate batch process
We prepared six microplates (576 samples) from stored blood spots, including .60 samples from known abnormal cases. Most of the prepared samples were analyzed twice to generate sufficient data for 1000 consecutive injections. Only for this experiment did we deviate from our routine conditions (see above) to use a faster flow rate (constant at 40 mL/min) and a faster injection cycle (one sample every 1.3 min). Also, the electrospray source temperature was increased to 150°C, to accord with the greater flow rate of the solvent. For recording multiple sample results in an easily visible format, we used an 8-s repetitive cycle containing four scan functions to generate a single continuum data file for acylcarnitines, two groups of amino acids, and free carnitine. The time allocated to each function within the cycle was apportioned according to relative ion intensity considerations within the analyte groups monitored [33].
computer algorithm for data processing
Selecting a set of control samples. The parameters to be used by the algorithm were selected by comparing the metabolic profiles from several known cases of organic acidemias and amino acid disorders with the profiles from the control samples. A large set of data files (n 5 1100) from our newborn population was chosen for establishing control cutoff values for the selected parameters. The criteria for this sample set were as follows: (a) samples from newborn infants who had a minimum birth weight of 2.01 kg; (b) samples analyzed in ,72 h from time of blood collection; and (c) data files designated, after manual scrutiny by expert analysts, as “not remarkable.”
Algorithm design and architecture. We used a specialized data-processing algorithm, Computer-Assisted Metabolic Profiling Algorithm (CAMPA), to process the batches of MS/MS data files acquired in the routine mode of analysis. Written in Microsoft Visual Basic, CAMPA is based on our previously published automated key strokes (macro) for data smoothing, measurement, and hardcopy [33] and is designed to allow automated flagging of abnormal profiles.
Upon execution, table definitions and parameter values needed for the analysis are downloaded from three primary tables, all of which are held in a centralized Access Database. The function of each table is as follows:
1) Acquired functions of diagnostic interest (FDI). This table contains user-defined minimum thresholds for the measured base peak intensity (intensity of the highest peak in the spectrum) of each acquired scan function.
2) Masses of diagnostic interest (MDI). This table, used to define the masses of diagnostic interest for each of the acquired functions, contains the molecular masses corresponding to free carnitine, all acylcarnitines known to be of diagnostic value, all amino acids detectable by this method, all stable isotope-labeled internal standards, and some of the acylcarnitine peaks observed after medication (e.g., phenylacetylcarnitine and benzoylcarnitine, which result from using phenylacetate and benzoate mixture in urea cycle disorders). By using user-defined flags, we can designate some of these masses as “must find” masses. The table also includes (M 1 1) Da isotope masses, corresponding to the more abundant peaks observed in the normal profile for acylcarnitines.
3) Peak ratios of diagnostic interest (RDI). All diagnostic peak ratios are defined in this table, along with maximum (and some minimum) cutoff values for each ratio. Blood concentrations are calculated for analytes for which stable isotope internal standards have been included, by using peak-height ratios and a concentration factor defined with each record. Cutoff values for these ratios have been defined in terms of a maximum and minimum molar concentration value. Because isotopelabeled analogs are not included (or available) for several analytes, peak-height ratios are defined relative to internal standard peaks within a similar mass range. Heightratios of certain pairs of analyte peaks are also defined. The cutoff thresholds used are expressed as maximum absolute values for each diagnostic ratio. The denominators of all calculated ratios correspond to masses designated “must find.” Once these three tables have been downloaded, the user is prompted to specify profile printout requirements. Available options allow printing of either all sample data, only sample data designated “abnormal,” only sample data designated “low quality,” or the combination of the latter two (all data designated “abnormal” or “low quality”). Database storage of all calculated diagnostic information for each sample can also be specified. To permit unattended overnight data acquisition and processing, one can select a timer function that allows the algorithm run to be set up for data not yet acquired. In this case, the user is asked to specify the complete file path specification of the data directory, together with the delay interval that should elapse before processing begins. If the timer function is not utilized, the data files can be selected individually or in highlighted groups by using a custom dialog window.
Automated processing of MS/MS data. The Masslynx operating and processing software provided with the instrument is driven by automated key strokes to access dataprocessing functions provided in this software. Data smoothing by successive iterations of a moving median algorithm (peak-width parameter 5 0.4 Da) on convergence serves to reduce the intensity of narrow data spikes to the background value. A second smoothing step, based on a Savitzky–Golay algorithm (peak-width parameter 5 1.1 Da), restores a smooth gaussian shape to the squareedged peaks produced by the moving median smoothing algorithm [33]. After background values are subtracted from the smoothed spectrum, the absolute intensity (height) of each peak is measured. Peak masses are assigned to the calculated centroid (point at which areas are equal on either side of it) of the upper 50% portion of each peak. The measured masses (to 4 decimals), and corresponding absolute peak intensities, are presented in a numerical table within Masslynx. This table is automatically copied to the Windows clipboard and then retrieved for arithmetic processing within CAMPA. The program applies a negative mass shift of 0.3 Da to all the measured mass values in the data array, rounds the shifted masses to integer values, and sorts and searches the data array for instances where two or more peaks are listed with the same nominal mass. These duplicate mass entries do occur on occasion, despite the smoothing methods described above. In case of duplicates, the entry with the greater intensity is retained and the remaining entry(ies) is discarded.
Data analysis. Subsequent to the capture of the processed data for the file under inspection, a four-step analysis phase is initiated:
1) The base peak intensities for each of the four profiles are calculated from the captured data tables and compared with the minimum threshold values, which have been loaded from the FDI table. A “low quality” flag is set for sample data having a base peak intensity below the threshold value for any function.
2) The captured data array is searched for all of the masses defined in the MDI table. When found, these masses are moved into working fields within the MDI table. If none of the masses designated “must find” are found in the data array, the sample data are flagged “low quality.” The acylcarnitines section of the data that have not been moved in the array is then searched for remaining peaks (not defined in the MDI) for which the intensity is .5% of the base peak height. Any such peaks, if found, are listed as “exception masses” in the output file corresponding to that sample.
3) Peak-height ratios are calculated from the data in the working fields of the MDI for all peak ratios defined in the RDI. Concentration values can then be calculated and threshold testing performed on the calculated peak ratio or concentration value, as defined in the RDI. “Abnormal”
flags are set for sample data giving values outside the defined thresholds.
4) The output log file is checked for any “low quality” or “abnormal” flags. Depending on the initial printout options selected, hard copies of the smoothed analog profiles are automatically generated by the CAMPA keystroke-driven Masslynx software. Finally, a formatted report is printed, listing the samples flagged as “abnormal” or “low quality” and detailing the reasons for each flag. Also listed are those samples flagged to indicate detection of “exception masses.”
Results
ms/ms analysis
The rationale for the MS/MS scanning functions used for acylcarnitines and neutral and basic amino acids was previously described [26, 27, 29–33]. In the present study, another function, neutral loss of 56 Da, was added specifically to measure glycine. We adopted this function to produce a signal for the protonated molecular ion at m/z 132 that would be more abundant than the signal for the loss of 102 Da, described for other neutral amino acids. Labeled glycine (1-[13C-15N]glycine), used as the internal standard, exhibited the same loss of 56 Da that is indicative of the loss of the elements of butene (C4H8) from the butyl ester moiety for both unlabeled and labeled glycine (data not shown). Nonetheless, glycine determination was somewhat problematic. Although this approach allowed us to diagnose two cases of nonketotic hyperglycinemia, numerous screening samples gave high glycine values that were not reproducible when analyzed the next day. Additional work is underway to investigate the reason for this phenomenon. Until this issue is resolved, a sample with a glycine value above the cutoff value must be reanalyzed. If an initial high result is reproduced, we request a sample of cerebrospinal fluid (CSF) for glycine determination by both MS/MS and HPLC. Only when a high CSF/blood glycine ratio (.0.04) is obtained by both techniques, and the patient presents the expected clinical picture of nonketotic hyperglycinemia, should a preliminary diagnosis of this disorder be made [37].
microplate batch process and sample
throughput
In the new microplate batch process we developed, 96 samples per batch could be prepared in ;2.5 h, a major improvement over previously published methods [31–33], which use three vials (or tubes) per sample and require labor-intensive labeling, capping, uncapping, and individual transfer of extracts between vials. In a qualitative experiment we estimated the biological load that the electrospray interface can accommodate without loss of data quality. Fig. 1 shows the first (A) and last (B) 200 min of the acylcarnitines profile from a sample run comprising data from 1000 blood-spot extracts, prepared by the microplate batch process for one 4.75-mm-diameter punch from each blood spot. Because this experiment was not practical at our routine injection interval time of 2.7 min (i.e., total run time would have been 45 h), we used a faster injection cycle and flow rate. Visual examination of the profiles indicated very little loss in sensitivity between the beginning and end of this 1300 min (;22 h) run. Profiles for the other functions monitored simultaneously were of equal quality (data not shown). Fig. 2 shows the abnormal acylcarnitines profiles obtained for a patient with glutaric acidemia type-II (GA-II), from whom a processed blood spot was injected at 494 min and repeated at 1258 min into the run. Comparison of the two profiles illustrates the high consistency (reproducibility) in spectral quality achieved with our method throughout this protracted multiple-sample run.
determination of newborn population
reference values
The algorithm developed (CAMPA) was initially used to process and quantify selected parameters from a group of .1000 profiles, which had been acquired individually as separate data files under our routine acquisition conditions (multichannel analysis mode and 2.7-min cycles) and classified as “normal” by manual scrutiny. We made no assumptions as to the nature of the distribution of the analyte concentrations or peak ratios, although for most the mean and median were very close. To determine cutoff values for these analytes, we used the percentile method [38]. Accordingly, the values obtained for each analyte were ranked, and the 0.5th and 99.5th percentiles were calculated. The 99.5th percentile was set as the high limit for each of the parameters. For some key metabolites a lower concentration limit, corresponding to the 0.5th percentile, was also used. The cutoff values obtained for amino acids and acylcarnitines were then plugged into CAMPA.
Table 1 shows the blood concentration and cutoff values for key amino acids in our newborn population. The mean blood concentration for phenylalanine in our study was 72 6 21 mmol/L, in agreement with literature values for newborns both by FAB-MS/MS and by HPLC [29, 39, 40]. A phenylalanine cutoff value of .129 mmol/L (99.5th percentile) is very close to the internationally recognized value of 120 mmol/L (2 mg/dL) used in many PKU (or hyperphenylalaninemia) screening programs[10, 12].
The mean concentration for tyrosine was 100 6 46 mmol/L, also similar to the 82 6 20 mmol/L reported in newborns [39]. The mean concentration for methionine was 28 6 10 mmol/L, quite close to the 31 6 8 mmol/L determined by HPLC for 11-day-old infants reported by Scott et al. [39], and an upper cutoff value of 59 mmol/L for blood methionine obtained in this study agreed favorably with the 67 mmol/L (1 mg/dL) used in screening for isolated hypermethioninemia and homocystinuria in some programs [13, 31].
Valine concentration in our newborn population was 116 6 36 mmol/L, compared with 111 6 24 mmol/L determined by HPLC [39] and with 131 6 58 mmol/L by FAB-MS/MS [30]. Our value of 103 6 33 mmol/L for leucine (1 isoleucine) was less than the literature values reported for the other methods [30, 39]. The mean blood concentration of glycine, however, 402 6 111 mmol/L, was higher than the 191 6 96 mmol/L reported by Scott et al. [39].
Table 1 also presents average abnormal concentrations and ranges for diagnostic metabolites in known cases as they pertain to certain amino acid disorders. The data for some disorders are combined when the biochemical marker is the same, e.g., for homocystinuria/hypermethioninemia and for tyrosinemia type I/type II. In tyrosinemia type I, methionine may also be substantially increased; however, both biochemical markers are not sufficiently diagnostic for the disease [33].
Some other diagnostic parameters based on peakheight ratios of pairs of analytes are also included in Table 1. The peak ratio of citrulline to tyrosine acquired in the scan function for neutral loss of 119 Da was extremely useful for detecting several cases of argininosuccinic synthetase deficiency and argininosuccinase deficiency
[33]. A peak ratio of the less abundant deammoniated butyl citrulline (m/z 215) to phenylalanine (in the neutral loss of 102 Da scan function) was also useful for argininosuccinic synthetase deficiency. Furthermore, we have
diagnosed two cases of hyperprolinemia by measuring the peak-height ratio of proline (m/z 172) to phenylalanine. However, proline was also somewhat increased in some cases of liver dysfunction. Other ratios of less diagnostic power were those for pyroglutamic acid (pipecolic; m/z 186) to phenylalanine, used in the diagnosis of pyroglutamic acidemia or pipecolic acidemia [33].
Most of the diagnostic parameters obtained from acylcarnitines were peak-height ratios relative to a known amount of an internal standard (usually d3-octanoylcarnitine, d3-C8), or peak-height ratios of some analyte pairs. Table 2 shows a description of each of these diagnostic ratios and provides a comparison between the upper cutoff value and the average abnormal value for each marker as they pertain to different disorders. The mean blood concentration of free carnitine in our unaffected newborn population was 22 6 8 mmol/L, which is intermediate between concentrations of 15 6 4 mmol/L in plasma isolated from cord blood and 34 6 5 mmol/L for plasma from children (ages 2 months to 5 years) as reported by Bhuiyan et al. [19]. The lower and upper cutoff values for free carnitine determined by our method were 9 and 59 mmol/L, respectively. This parameter is not included in Table 2 because it varies significantly with disease, metabolic status of the patient, and treatment. Most of the diagnostic parameters, however, showed a clear distinction between the abnormal value obtained from patients with the metabolic disorder and the upper cutoff value from the population of unaffected newborns.
testing the discriminative power of campa
Two retrospective experiments were carried out to verify the performance of CAMPA and to quantify the specificity and sensitivity of the algorithm before introducing it into unchecked daily service in our laboratory. The first test consisted of 559 MS/MS data files that had been compiled in one directory on the processing PC. Among these were 119“abnormal”profiles obtained from patients with known metabolic diseases who had been diagnosed from MS/MS results and who exhibited clini-
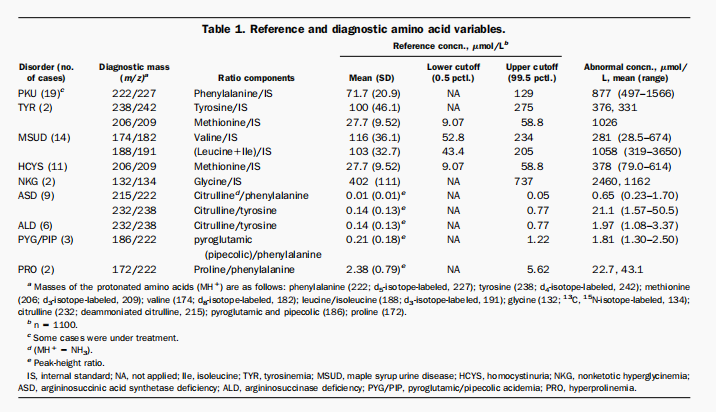
cal symptoms; many of these samples were from patients undergoing treatment at the time of sample collection. The remainder (440) were“normal”profiles from the neonatal screening samples. The second test involved a larger data set of 1151 files: 147“abnormal”files (including the previous 119 files) and a new batch of 1004“normal”data files from neonatal screening samples. CAMPA was applied to both data sets, and summary reports were generated and compared with previously obtained manual findings. Collectively, the results indicated that the sensitivity of the algorithm was 100% and the weighted average cumulative specificity for the two tests was 83.1% (Table 3).
A flow chart (Fig. 3) summarizes the processes that occurred when CAMPA was launched for a selected data set. Fig. 4 shows an example of the output of the computer algorithm, i.e., an abnormal acylcarnitine spectrum for a case of medium-chain acyl-CoA dehydrogenase deficiency diagnosed by this technique in neonatal screening samples. The output report for this sample, as determined by CAMPA, is pasted to the spectrum. Basically, four abnormally increased species are evident in the spectrum: octanoylcarnitine (C8; m/z 344), hexanoylcarnitine (C6; m/z
316), decanoylcarnitine (C10; m/z 372), and decenoylcarnitine (C10:1; m/z 370) [33, 41, 42]. As presented in the spectral data and the abnormality details, the most outstanding increase was in the concentration of C8, which was 22-fold above the upper normal value in our newborn population. C6, C10, and C10:1 were also above the respective threshold values.
Discussion
The introduction of ESI-MS/MS as a diagnostic tool in our metabolic unit has had a great impact on the number of cases diagnosed as organic acidemias or aminoacidopathies from referred sick children [32–34]. The reliable semiquantitative data (based on isotope-dilution mass spectrometry), reproducibility, and rapid turnaround time of the technique have made it a preferred method for clinical follow-up—particularly for management of amino acid disorders, such as PKU, maple syrup urine disease, and hyper- and hypomethioninemia. We continue to confirm MS/MS results by more-conventional methods, e.g., GC/MS for determination of urinary organic acids, HPLC for plasma or CSF amino acids, and enzymatic assay when available.
Our main objective in the current study was to take further steps toward establishing ESI-MS/MS as a robust, high-sample-throughput, and efficient neonatal screening method. Our first goal was to increase sample throughput by simplifying sample preparation. We converted the sample preparation to a more efficient and cost-effective batch-type process by using the convenience of 96-well microplates. Automated punching and microplate mapping eliminated the tiresome process of labeling individual sample vials and writing lists of sample names. The inherent possibilities for sample confusion within these processes have accordingly been substantially reduced. Generally, two technicians can now prepare six microplates (576 samples) in a regular working day. In addition, the use of one 4.75-mm (7 mL) blood-spot punch reduced by half the biological load of sample injected into the ion source of the mass spectrometer.
This made it practical to inject 500 samples per day into an instrument for our routine cycle of 2.7-min intervals between injections (total: 1350 min or 22.5 h) and to acquire each sample as a separate data file. We foresee that the whole process could be fully automated by using modified microplate robotics, which might further reduce the cost of labor and improve precision.
Data interpretation by manual scrutiny is a laborintensive and subjective process requiring considerable experience and expertise. The algorithm, CAMPA, was developed to transform the interpretation process into an objective, efficient, quantitative, and automated process.
Designed as a flexible program, CAMPA is totally configured via the parameter setup in the database tables. This allows an expert user to change the concentrations of the internal standards added during sample preparation, whereby all formulas for metabolite concentrations are then automatically adjusted. In addition, CAMPA can easily allow addition, or elimination (or inactivation), of various markers as more information is acquired from new metabolic cases and from larger data sets for our newborn population.
Several criteria were extremely important for achieving the goals for which the algorithm was developed. The first criterion was to provide the algorithm with high-quality MS/MS data, free from detector artifacts that might otherwise be designated as metabolite peaks, and with no errors in mass assignments. In this regard, introduction of the combined median and Savitsky–Golay smoothing techniques proved useful for eliminating detector spikes from the raw spectra, nullifying the potential effects of these spikes on the relative heights of the diagnostic peaks, without substantially affecting peak width. In
Our previous work with acylcarnitines, we noted that integer mass assignments were sometimes in error by a 1-Da excess; the Masslynx software had generated these integers by rounding off measured values (i.e., 347.50 would become 347 and 347.51 would become 348). Instrument considerations, peak asymmetry, and low ion counts can produce a measured mass accuracy error of ;6 0.2 Da in the longer-chain acylcarnitines. Considering that the calculated monoisotopic mass of the butyl ester of palmitoyl-carnitine is 456.4 Da, rounding measured values might occasionally assign the ester an integer mass of 457. To overcome these inconsistencies, we used a “Delta” mass shift facility (provided in the Masslynx peak annotation software) to apply a 0.3-Da negative mass shift to all measured masses before rounding them off. In designing the analytical mechanism of CAMPA, we decided to incorporate a delta mass shift, similar to that used in the printout annotation, within the data-processing structure of CAMPA. This process has also been shown to give correct integer values for masses in the amino acids profiles. Mass entries in the MDI and RDI tables were also made as integer values. The unique numerical values for diagnostic masses and concurrence with printout annotation provided by this system have simplified interpretation of CAMPA output and discussion of profile abnor-malities.
Another important criterion for CAMPA was to provide it with adequate parameters to be tested. Successful selection of these parameters was possible because of the large number of metabolic cases in our files and the wealth of data available in the literature. Selection of flagging markers for amino acid disorders was straightforward, usually depending on the increase of one or more amino acids, e.g., methionine in hypermethioninemia/homocystinuria, and leucine (1 isoleucine) and valine for maple syrup urine disease. In some diseases, the variability of the data could be reduced by establishing markers as a ratio of one amino acid to another, e.g., the phenylalanine/tyrosine ratio for hyperphenylalaninemia, and methionine/leucine for hypermethioninemia [13, 15]. The situation is not as simple for organic acidemias and fatty acids oxidation defects. Although for some diseases a certain metabolite is the key in making a preliminary diagnosis, e.g.,
increased glutarylcarnitine in glutaric acidemia type I (GA-I), for most of these disorders several metabolites were found to be increased. For example, in GA-II (Fig. 2), in medium-chain acyl-CoA dehydrogenase deficiency (Fig. 4), and in long-chain (or very-long-chain) acyl-CoA dehydrogenase deficiency, several acylcarnitines were increased above normal values [33, 41, 42]. In addition, for most of these disorders, measurement of free carnitine is important in the initial blood sample and in posttreatment follow-up samples. Accordingly, many parameters had to be selected and cutoffs established for these disorders (see Table 2). Unlike the amino acids, the concentration of some of the diagnostic acylcarnitines in normal blood samples is often below the detection limit of the method, e.g., glutarylcarnitine (C5-dicarboxylic), diagnostic for GA-I; 3-methylglutarylcarnitine (C6-dicarboxylic), diagnostic for 3-hydroxy-3-methylglutaryl-CoA-lyase deficiency; and tiglylcarnitine (C5:1), which is of diagnostic value in b-ketothiolase deficiency [26, 33]. For this reason, we applied lower cutoff limits only to those metabolites that were consistently present in spectra of normal samples, such as free carnitine, acetylcarnitine, and palmitoylcarnitine.
Determination of population-based cutoff values from a large number of normal samples was another important criterion toward achieving high reliability in discernment of abnormal profiles. Although CAMPA is being used for both selective and neonatal screening for metabolic diseases, the population selected for analysis to determine cutoff values was normal (unaffected) neonates. The decision to use values from newborn blood samples was made because of the availability of a large number of samples from this population, and because this is our target group for a neonatal screening program. The ability to use CAMPA to calculate and store all values obtained from a large data set (n 5 1100) allowed us to establish reliable cutoff values for the selected markers. For the most part, values obtained for key blood metabolites by ESI-MS/MS, particularly for the amino acids, are very similar to those obtained by conventional techniques. The success of the algorithm has been evident in the absolute sensitivity of CAMPA for flagging cases with known metabolic disorders. As for the difference between the sensitivity and the specificity of the test (,17%), we found that variability in the acylcarnitine values was responsible for .85% of “false flagging.” In particular, propionylcarnitine and butyryl (or isobutyryl)carnitine ratios just above the respective cutoff values were responsible for ~25% of the falsely flagged samples, whereas in reality these minor increases are of no pathological significance. Of the falsely flagged data, <5% was due to borderline increase of one or more of the key amino acids such as methionine, leucine (1 isoleucine), alanine, glycine, and phenylalanine. The remaining 10% were the result of borderline low values for one or more of these amino acids. In most falsely flagged data files, a single parameter was usually the reason the flag was set. In most “truly abnormal” data files, however, multiple flags were set, and the values obtained for concentrations or ratios were noticeably higher than the cutoff values (see Tables 1 and 2). Routinely, the analyst examines the report detailing the reasons for flagging each data file and visually inspects the spectra for these files, thereby permitting an accurate diagnosis. He or she then decides whether to repeat analysis of the sample or to annotate it as “not remarkable.” Because of the severity of many of these diseases, a report would immediately be sent out to the clinician, for any patient whose profiles were clearly abnormal, as determined by CAMPA, followed by expert concurrence. Also, to assure reproducibility of the initial results, the sample would be reanalyzed in the next batch of samples.
In developing a new screening method or program, the probability of both false-positive and false-negative results should be rigorously tested. A high rate of falsepositives will both increase the cost of the screening program and put an emotional burden on the parents, when they are requested to submit repeat samples. As pointed out by Chace et al. [31], and by the work presented here, the specificity and accuracy provided by MS/MS over conventional screening methods should reduce the rate of false-positives and therefore reduce the number of repeat samples. False-negatives are even more costly: They involve missing cases of possibly treatable disorders and could seriously undermine the screening program. In this study, the calculated sensitivity for the method was based on the results for known metabolic cases and, to the best of our knowledge, we have not encountered a false-negative case. However, additional time and more testing are required to further ensure the reduction or elimination of false-negatives. In this regard, development of collaborative interlaboratory quality-assurance programs appears necessary to further validate the method.
In conclusion, the development and use of CAMPA and the microplate batch process has allowed all laboratory personnel in our metabolic unit to proceed with a batch of samples from initial sample registration through production of a final printed output report. The introduction of CAMPA has provided our expert analysts with a powerful quantitative tool for detecting abnormal profiles and has substantially decreased the time spent in manual scrutiny of multiple spectra for each patient’s sample.
These new facilities have given the unit a high-through-put capacity, allowing analysis of hundreds of samples on a daily basis. We believe that these advances in metabolic profiling methodology, and the resulting cost-effectiveness per sample, make ESI-MS/MS the method of choice for establishing neonatal screening programs for an important group of metabolic diseases. In this regard, our laboratory has recently started a pilot neonatal screening program for this category of diseases in Saudi Arabia.
We express our deepest gratitude to Dr. Sultan Al Sedairy, Executive Director, of the Research Centre of King Faisal Specialist Hospital and Research Centre (KFSH & RC), Riyadh, for his continuous support of our metabolic unit. We are grateful for the administration, clinicians, and nurses of several hospitals, in particular Prince Salman Hospital, Riyadh, for their significant contribution of the neonatal sample material. We also thank John Crawford, of the Cyclotron Operations Section of the Research Centre of KFSH & RC, for his contributions to the design and mechanical construction of our automated blood-spot puncher.
2. Moammar H, Ratard R, Cheriyan G, Mathew P. Incidence and features of galactosemia in Saudi Arabs. J Inher Metab Dis 1996;19:331–4.
3. Teebi A. Autosomal recessive disorders among Arabs: an overview from Kuwait. J Med Genet 1994;31:224–33.
4. Temtamy SA, Kandil MR, Demerdash AM, Hassan WA, Meguid NA, Afifi HH. An epidemiological/genetic study of mental subnormality in Assiut Governorate, Egypt. Clin Genet 1994;46:347–51.
5. Arn PH, Valle DL, Brusilow SW. Inborn errors of metabolism: not rare, not hopeless. Contemp Pediatr 1988;5:47–63.
6. Ward JC. Inborn errors of metabolism of acute onset in infancy. Pediatr Rev 1990;11:205–16.
7. Goodman SI. Inherited metabolic disorders in the newborn: approach to diagnosis and treatment. Adv Pediatr 1986;33:197– 224.
8. Leonard JV. The early detection and management of inborn errors presenting in the neonatal period. Eur J Pediatr 1985;143:253–7.
9. Seashore MR, Rinaldo P. Metabolic disease of the neonate and young infant. Semin Perinatol 1993;17:318–29.
10. Seashore MR. Neonatal screening for inborn errors of metabolism: update. Semin Perinatol 1990;14:431–8.
11. Scriver CR. Genetic screening, testing and treatment: how far can we go? J Inher Metab Dis 1996;19:401–11.
12. Guthrie R, Susi A. A simple phenylalanine method for detecting phenylketonuria in large populations of newborn infants. Pediatrics 1963;32:338–43.
13. Guthrie R. Screening for “inborn errors of metabolism” in the newborn infant—a multiple test program. Birth Defects 1968;4: 92–8.
14. Turnell DC, Cooper JDH. Rapid assay for amino acids in serum or urine by pre-column derivatization and reversed-phase liquid chromatography. Clin Chem 1982;28:527–31.
15. van Eijk HMH, van der Heijden MAH, van Berlo CLH, Soeters PB. Fully automated liquid-chromatographic determination of amino acids. Clin Chem 1988;34:2510–3.
16. Bremer J. Carnitine—metabolism, functions. Physiol Rev 1983; 63:1420–80.
17. Stanley CA. Carnitine disorders. Adv Pediatr 1995;42:209–42.
18. Chalmers RA, Roe CR, Stacey TE, Hoppel CL. Urinary excretion of L-carnitine and acylcarnitines by patients with disorders of organic acid metabolism: evidence for secondary insufficiency of L-carnitine. Pediatr Res 1984;18:1325–8.
19. Bhuiyan AKMJ, Jackson S, Turnbull DM, Aynsley-Green A, Leonard JV, Barlett K. The measurement of carnitine and acylcarnitines: application to the investigation of patients with suspected inherited disorders of mitochondrial fatty acid oxidation. Clin Chim Acta 1992;207:185–204.
20. Minkler PE, Hoppel CL. Quantification of free carnitine, individual short- and medium-chain acylcarnitines, and total carnitine in plasma by high-performance liquid chromatography. Anal Biochem 1993;212:510–8.
21. Schmidt-Sommerfeld E, Penn D, Duran M, Bennett MJ, Santer R, Stanley CA. Detection of inborn errors of fatty acid oxidation from acylcarnitine analysis of plasma and blood spots with the radioisotopic exchange-high-performance liquid chromatographic method. J Pediatr 1993;122:708–13.
22. Kodo N, Millington DS, Norwood DL, Roe CR. Automated analysis of free and short-chain acylcarnitine in plasma with a centrifugal analyzer. Clin Chem 1992;38:2215–21.
23. Van Bocxlaer JF, De Leenheer AP. Solid-phase extraction technique for gas chromatographic profiling of acylcarnitines. Clin Chem 1993;39:1911–7.
24. Lowes S, Rose ME, Mills GA, Pollitt RJ. Identification of urinary acylcarnitines using gas chromatography–mass spectrometry: preliminary clinical applications. J Chromatogr 1992;577:205–14.
25. Minkler PE, Hoppel CL. Quantification of carnitine and specific acylcarnitines by high-performance liquid chromatography: application to normal human urine and urine from patients with methylmalonic aciduria, isovaleric acidemia or medium-chain acyl-CoA dehydrogenase deficiency. J Chromatogr 1993;613:203–21.
26. Millington DS, Kodo N, Norwood DL, Roe CR. Tandem mass spectrometry: a new method for acylcarnitine profiling with potential for neonatal screening for inborn errors of metabolism. J Inher Metab Dis 1990;13:321–4.
27. Millington DS, Kodo N, Terada N, Roe CR, Chace DH. The analysis of diagnostic markers of genetic disorders in human blood and urine using tandem mass spectrometry with liquid secondary ion mass spectrometry. Int J Mass Spectrom Ion Processes 1991; 111:211–28.
28. Millington DS, Terada N, Chace DH, Chen Y, Ding J, Kodo N, et al. New developments in fatty acid oxidation. In: Coates PM, Tanaka K, eds. The role of tandem mass spectrometry in the diagnosis of fatty acid oxidation disorders. New York: Wiley–Liss, 1992:339– 54.
29. Chace DH, Millington DS, Terada N, Kahler SG, Roe CR, Hofman LF. Rapid diagnosis of phenylketonuria by quantitative analysis for phenylalanine and tyrosine in neonatal blood spots by tandem mass spectrometry. Clin Chem 1993;38:66–71.
30. Chace DH, Hillman SL, Millington DS, Kahler SG, Roe CR, Naylor EW. Rapid diagnosis of maple syrup urine disease in blood spots from newborns by tandem mass spectrometry. Clin Chem 1995; 41:62–8.
31. Chace DH, Hillman SL, Millington DS, Kahler SG, Adam BW, Levy HL. Rapid diagnosis of homocystinuria and other hypermethioninemias from newborns’ blood spots by tandem mass spectrometry. Clin Chem 1996;42:349–55.
32. Rashed MS, Ozand PT, Harrison ME, Watkins PJF, Evans S. Electrospray tandem mass spectrometry in the diagnosis of organic acidemias. Rapid Commun Mass Spectrom 1994;8:129–33.
33. Rashed MS, Ozand PT, Bucknall MP, Little D. Diagnosis of inborn errors of metabolism from blood spots by acylcarnitines and amino acids profiling using automated electrospray tandem mass spectrometry. Pediatr Res 1995;38:324–31.
34. Attia N, Sakati N, Al Ashwal A, Alsaif R, Rashed M, Ozand PT. Isovaleric acidemia appearing as diabetic ketoacidosis. J Inher Metab Dis 1996;19:85–6.
35. Shigematsu Y, Hata I, Nakai A, Kikawa Y, Sudo M, Tanaka Y, et al. Prenatal diagnosis of organic acidemias based on amniotic fluid levels of acylcarnitines. Pediatr Res 1996;39:680–4.
36. Rashed MS, Ozand PT, Bennett MJ, Barnard JJ, Govindarju DR, Rinaldo P. Inborn errors of metabolism diagnosed in sudden infant death cases by acylcarnitine analysis of postmortem bile. Clin Chem 1995;41:1109–14.
37. Hamosh A, Johnston MV, Valle D. Nonketotic hyperglycinemia. In: Scriver CR, Beaudet AL, Sly WS, Valle D, eds. The metabolic and molecular bases of inherited disease, 7th ed. New York: McGrawHill, 1995:1337–48.
38. Solberg HE. Establishment and use of reference values. In: Burtis CA, Ashwood ER, eds. Tietz fundamentals of clinical chemistry. Philadelphia: WB Saunders, 1996:182–91.
39. Scott PH, Sandham S, Balmer SE, Wharton BA. Diet-related reference values for plasma amino acids in newborns measured by reversed-phase HPLC. Clin Chem 1990;36:1922–7.
40. Caruso U, Cerone R, Fantasia AR, Schiaffino MC, Zignego G, Romano C. Plasma amino acids during the first 24 h of life: feasibility of early diagnosis in the newborn at risk of amino acid disorders. J Inher Metab Dis 1989;12(Suppl):311–4.
41. Van Hove JLK, Zhang W, Kahler SG, Roe CR, Chen Y, Terada N, et al. Medium-chain acyl-CoA dehydrogenase (MCAD) deficiency: diagnosis by acylcarnitine analysis in blood. Am J Hum Genet 1993;52:958–66.
42. Ziadeh R, Hoffman EP, Finegold DN, Hoop RC, Brackett JC, Strauss AW, et al. Medium chain acyl-CoA dehydrogenase deficiency in Pennsylvania: neonatal screening shows high incidence and unexpected mutation frequencies. Pediatr Res 1995;37:675–8.